Introduction of Completion fluids
Completion fluids can be defined as any drilling fluid pumped into oil and gas reservoir to conduct operations after the initial drilling of a well. The fluids can improve well productivity by reducing damage to the producing zone, and they can help prepare, repair, clean out, and complete the wellbore during the completion phase. Many drilling completion engineers now consider the ‘completion operation’ to begin as soon as the drill bit enters the productive interval. It is therefore necessary to plan procedures and implement practices to reduce formation damage, protect the reservoir, ensure downhole safety, smooth drilling work, and to maximize productivity at the earliest possible stage.
The water-based type is main category of completion fluid, which is most widely used at present. Completion fluids are typically brines (chlorides, bromides, and formates). Nearly all completions are run with a clear, solid-free brine in the well. For many completion operations, a brine column is the primary well control barrier. Where brine has a well control function, it must be dense enough to overbalance any formation pressure by a margin sufficient to prevent an influx. Since brine is nominally solid-free, any overbalance pressure acting against a permeable formation will result in fluid loss to the formation. In order to perform the desired function, completion fluids must control formation pressure, protect the productive zone, be stable under surface and downhole conditions, be safely handled, be environmentally friendly or used with controlled exposure, and be cost effective.
Different types and properties of completion fluids are required for different oil and gas reservoirs and different drilling engineering requirement. Proper selection and application of the completion fluid is an integral part of this process. No matter what completion fluids are chosen, the basic requirements are similar: controlling the formation fluid pressure and ensuring normal drilling. The completion fluid density can be regulated in accordance with actual downhole conditions. Density and solid content are typical performance specification for clear brine. Proper density is necessary for pressure control. Clarity is necessary to eliminate formation plugging by solids. In order to select a completion fluid appropriate for the application, one must understand the various types and properties of clear brine fluids.
OLI Systems simulation for completion fluids
Thermodynamic simulations are essential for designing the properties of completion fluids, modeling the pressure, protecting the reservoir, maximining the productivity and lowering cost. OLI systems has developed a comprehensive thermodynamic model to predict the properties needed to model the completion fluids. OLI software V11 is based on OLI’s proprietary Mixed Solvent Electrolyte (MSE) thermodynamic model (1). The properties include relevant brine solubilities, densities (with their dependence on temperature and pressure), vapor-liquid-solid phase equilibrium, enthalpies, and heat capacities for mixed brines. The properties cover a wide range of temperature, pressure and composition that are needed model the completion fluids. Several diagrams are given that compare the MSE model predictions to experimental data demonstrating the accuracy of the model in capturing some of these key properties. OLI Systems V11 is sufficiently robust and extendible to deliver rigorous and accurate chemistry simulation for any complex and interactive chemistry of interest.
Brine selection
Where brine forms the primary well control barrier, the first step in selecting a brine is to calculate the density needed to overbalance formation pressure. Once brine density has been calculated, it must be adjusted to correct for wellbore average temperature and pressure. With this done, a suitable brine can be selected. Table 1 shows that OLI platform V11 lists the most common types of brines.
Table 1. Brines in OLI platform V11
Brine type | Formula | Brine type | Formula |
Ammonium chloride | NH4Cl | Sodium bromide | NaBr |
Potassium chloride | KCl | Sodium chloride/bromide | NaCl/NaBr |
Sodium chloride | NaCl | Calcium bromide | CaBr2 |
Potassium bromide | KBr | Calcium chloride | CaCl2 |
Calcium chloride/bromide | CaCl2/CaBr2 | Zinc bromide | ZnBr2 |
Sodium formate | NaCOOH | Cesium formate | CsCOOH |
Sodium acetate | C2H3NaO2 | Cesium acetate | C2H3CsO2 |
Brine density
Since the brine provides a well control barrier, calculating the correct brine solution density is crucial. Density calculations will always be the first step in the brine selection process. The brine must have sufficient density to exceed formation pressure by the required margin. Its density is directly related to the salt concentration and the proportions of various salts. Density varies with temperature, and to a lesser extent, pressure. Increasing temperature causes fluids to expand, with a consequent decrease in density. Increasing pressure causes a density increase.
Because pressure is cumulative with the depth of the well and is directly related to density, it is necessary to mathematically predict the density of the completion fluid under the combined influence of compression and temperature. Figure 1 shows the density behavior on NaBr in water as a function of pressure up to 1500 atm at various concentrations (0.22-7.61 mol/kg or 2.24-43.9% weight percent) and at a high temperature of 200 °C. Figure 1 indicates a good agreement of the prediction with experimental data. OLI Software Platform V11 not only predicts the density behavior of all the brines listed in table 1, but also calculates other properties of brines solutions (activity of water and activity coefficient of aqueous brines, vapor-liquid equilibria, enthalpies, and heat capacities of the solution).
Crystallization temperature
In addition to density requirements, brine selection must also take into account the true crystallization temperature (TCP). The crystallization temperature of a brine is the temperature at which solids start to form. High-salinity salt solutions close to a saturation condition under a higher temperature will be able to reach saturation or a supersaturated condition when temperature is reduced to a certain value. Those solids will either be ice crystals formed by the freezing of water, or salt crystals forming as brine salts come out of the solution. Salt crystals can plug lines, filtration units and interfere with pumping operations. Of greater concern is the potential for crystallization to result in a reduction of the brine hydrostatic pressure, leading to a well control incident. In such event, the completion fluid does not meet the design requirement and cannot be used. Knowing the brine crystallization temperature is therefore essential.
Solubility cures are commonly used to illustrate the relationship between solubility, temperature, and solvent type. Once an appropriate solvent is chosen, the solubility curve becomes a critical tool for the development of an effective crystallization process. Figure 2 shows the solubility behavior of cesium acetate in water as a function of temperature ranging from approximately -80 to 120 °C and indicates a good agreement of the predictions with experimental data. Besides anhydrous cesium acetate, two solid cesium acetate hydrates exist, with 1 and 1.5 water molecules. These salts are very soluble in water. The calculation of the thermodynamic properties of cesium acetate salts and their mixtures is a challenge for aqueous solution modeling. Figure 2 also shows that as cesium acetate dissolves in the completion brine, the freezing point drops, and ice crystals begin to form in the brine. Dissolving more cesium acetate both increases the brine density and reduces the freezing point down to the eutectic pint (-80°C). It is important to know the salt solution crystallization temperature when the designed completion fluid is stored in cold weather or when it is used offshore where the seawater may be cold.
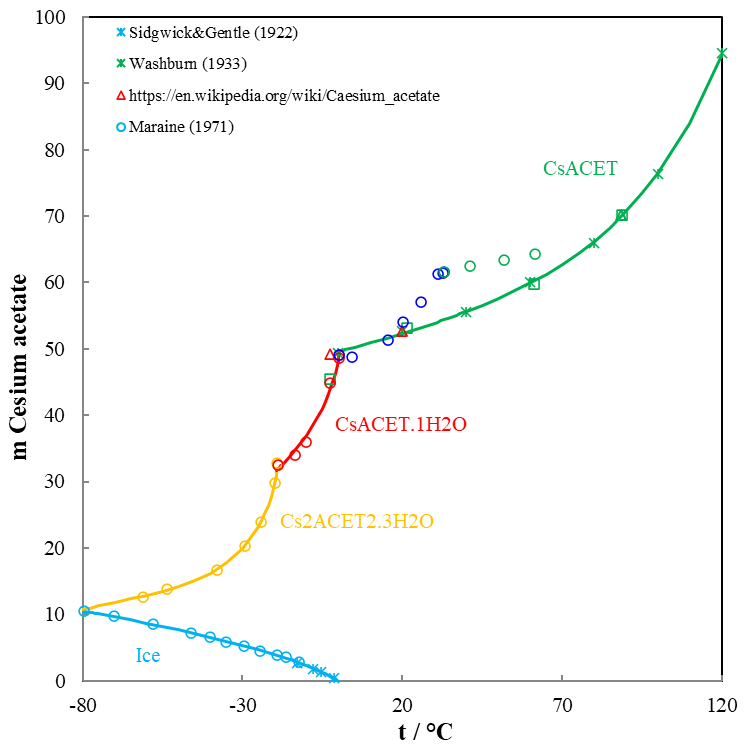
Crystallization temperature is related to the type of the salt and the proportions of different salts. Most single salt brines have qualitatively similar phase diagrams and the relationship between temperature and density is predictable. As a result, when the mixed salt is selected to adjust and control completion fluid density by varying the concentration of the different salts in solution, a multi-salt brine can be formulated with a wide range of crystallization temperatures. Figure 3 shows the solubility curve of the ternary system sodium acetate- cesium acetate-water. The MSE model reasonably predicts the formation of 4 precipitating solids, i.e., CH3COONa, CH3COOCs, CH3COOCs·1H2O and CH3COONa·3H2O. From 0 to 100 °C, the crystallization region of CH3COONa.3H2O becomes the largest and the region of CH3COONa becomes the smallest at 50 °C. On the other side, the crystallization region of CH3COOCs·1H2O disappears. At low temperature, 0 °C, the ternary system shows a complex solubility behavior. The results from the MSE model provide insights and a theoretical foundation for the crystallization temperature and concentration of mixed salts.
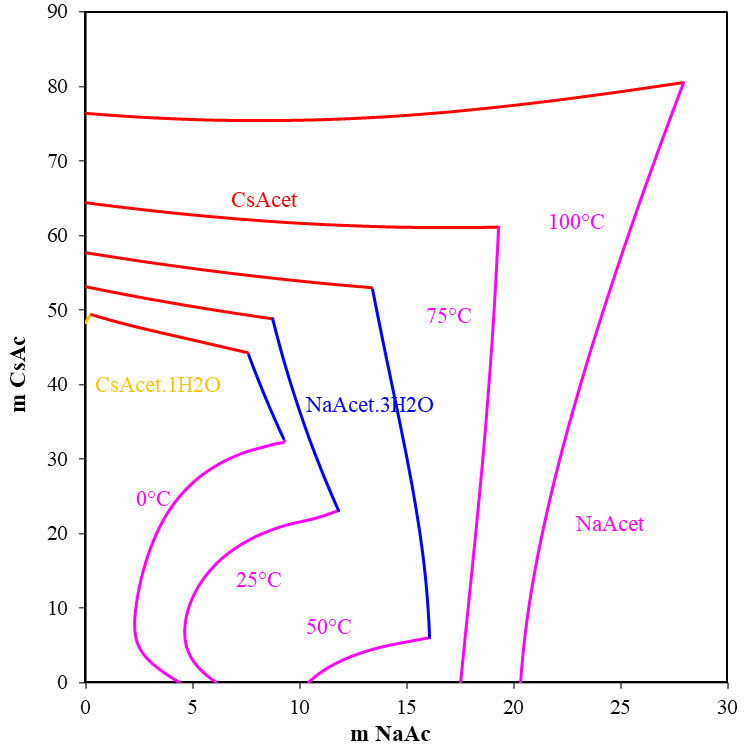
Brine crystallization temperature is typically measured at atmospheric pressure. At high pressures, the crystallization temperature increases. Especially in deep-water operations, a brine’s pressure-dependent crystallization temperature is important because of colder temperatures and higher pressures at the sea floor. In deeper water, crystallization is most likely to occur at the sea floor – typically the coldest point in the well. Therefore, accurate prediction of the solubility dependence on pressure is also essential to select a brine. As an example, Figure 4 shows the sodium chloride solubility in water at 25 °C from atmospheric pressure to 1500 atm. The MSE model is calibrated by reproducing selected experimental data in binary systems within their experimental uncertainty and then it can predict the behavior of the pressure effect on solubility.
Summary
The diagrams given above show that the MSE model can accurately predict the properties needed to model completion fluid chemistry over a wide range of temperature, pressure, and composition. Specifically, the model can predict the effect of temperature and pressure for the density and solubility of single and mixed brines. For the design and application of completion fluids, it is necessary to know different types and properties of brines, which can be obtained from OLI MSE model.
What tools are available for predicting completion fluid chemistry?
The OLI Systems software products – OLI Studio and OLI Flowsheet: ESP – use the OLI MSE thermodynamic model to accurately simulate completion fluid chemistry.
Contact OLI for more information or to schedule a meeting with an OLI expert.
References
- Wang P., Anderko A., Young R. D., “A Speciation – Based Model for Mixed – Solvent Electrolyte Systems”, Fluid Phase Equilibria, 203, (1-2), 141-176, 2002.